All published articles of this journal are available on ScienceDirect.
Biological Membranes and Malaria-Parasites
Abstract
Paludisme "a word derived from Latin palus meaning swamp" or Malaria " a word derived from Italian mala'ria meaning bad air", designed by the bad air from swamps, is an infectious disease caused by a parasite of the genus Plasmodium transmitted by female mosquitoes of the genus Anopheles generating millions of deaths each year. Biological membranes have a major role in cells invasion by Malaria parasites. Phosphatidylserine and phosphatidylinositol are essential for the invasion of erythrocytes by Plasmodium. Plasmodium binds to the erythrocyte membrane via glycolipids. Cholesterol is responsible for the uptake of host proteins and maintenance of intracellular parasitophorous vacuolar membrane. Malaria parasites invade red blood cells by binding to multiple membrane receptors at the level of the spectrin, band 3, actin, glycophorin, band 4.1, band 4.2, aquaporin-1, band 7, and ankyrin. Parasitic proteins such as the reticulocyte-binding like family bind to the membrane erythrocytic proteins and play a major role in the mechanisms of invasion of red blood cells by Plasmodium. Susceptibility to Plasmodium invasion is linked to the terminal stages of the differentiation of red blood cells. This review highlights the complex interactions between biological membranes and malaria parasites.
1. INTRODUCTION
Malaria is an infectious parasitic disease infecting about 500 million people and causing about 3 million deaths each year, according to the World Health Organization. About 3.3 billion people worldwide are exposed to malaria [1, 2]. Descriptions of the symptoms of this disease are found in the papyrus of Ebers and at Hippocrates [3-5]. Alphonse Laveron discovered the parasite Plasmodium in the erythrocytes of patients with malaria fever [6-8]. Malaria in humans is caused by four species of the genus Plasmodium which are Plasmodium falciparum, Plasmodium vivax, Plasmodium malariae and Plasmodium ovale. Plasmodium vivax and Plasmodium ovale emerge at the Pliocene. Plasmodium falciparum occurs in the Pleistocene. Plasmodium malariae is common to humans and chimpanzees [9, 10].
Plasmodium recognizes specific receptor sites on the surface of cells and enters these cells to develop. The nature of cells seems to influence the mechanisms of recognition or interaction between the parasite and its host cells. How can the structure of cells intervene in parasite invasion?
2. CYCLE OF PLASMODIUM
The genus Plasmodium of the family of Plasmodiidae of the Haemosporina suborder of the Telosporea class is characterized by schizogonies in the host receiver and sporogonies in the vector insect. When the Anopheles bites a malarious subject, it ingests Plasmodium gametocytes. Once entering the mosquitoes, gametocytes differentiate into gametes. The female gametocyte, macrogametocytes, becomes a macrogamete in the mosquito's stomach. The male gametocyte, microgametocytes, gives microgametes. The microgamete and macrogamete perform sexual reproduction to produce an ookinete. Between the intestinal epithelium and the basement membrane, the ookinete is surrounded by a cystic membrane and gives an oocyst. After a series of mitosis, the oocyst gives sporoblasts which produce sporozoites by cryptomitosis. The sporozoites migrate to the salivary glands of the mosquito [11, 12]. During a blood meal, the infected anopheline female mosquito inoculates sporozoites in the capillaries of the vertebrates host. About 1 to 30 minutes after their inoculation, the sporozoites infect the hepatocytes. After the ring and trophozoite stages, the sporozoite undergoes a pre-erythrocytic asexual division process, schizogony, to produce a schizont that releases 16-32 daughter merozoites invading blood cells [13, 14]. The erythrocytic cycle is 48 hours. It is an endocytosis with 3 stages: i) attachment of merozoite to the red blood cell ii) invagination of the red blood cell membrane iii) internalization of the parasite in the RBC, it's a trophozoite phase. The trophozoite phase produces a parasitophorous vacuole where schizogony generates several merozoite daughter cells [15]. Merozoites are released into the blood by the bursting of their host cells to start a new cycle [16, 17]. Some merozoites become microgametocytes or macrogametocytes. It is the sexual gametocytogony phase (Figs. 1 and 2).
3. PHYSIOPATHOLOGY OF MALARIA
The erythrocytic stages are responsible for the pathologies and symptoms of malaria including fevers, anemias, lesions in the organs (spleen, liver, brain, kidneys), and severe brain disease. The fever is related to the bursting of the parasitic RBC and the release into the blood circulation of the hemozoin, parasitic pigment, and various pyrogenic substances. The bursting of the schizonts and the liberation of the merozoites could partly explain the erythrocyte destruction. Hemolysis also affected the infected and non-infected red blood cells, with some infested cells being eliminated by the spleen [18]. An immune response with complement participation would also play a role in hemolysis. The hemolytic activity of the mononuclear phagocytic system of the spleen especially and autoimmunity are largely responsible for the destruction of healthy red blood cells, which are phagocytosed and destroyed at the same time as the parasitized erythrocytes [19-22]. For self-immunizing hemolysis, which may play an important role, for example, in rabbits infected by Plasmodium berghei ANKA, Topley et al. discovered a complement on the surface of non-parasitized erythrocytes [23, 24]. Malaria is responsible for hyperreactivity of the reticuloendothelial system, which leads to the lysis of even non-parasitic erythrocytes [25, 26]. The hemolysis observed during infestation could be explained by an increased serum phospholipase A2 activity as demonstrated in Plasmodium falciparum malaria [27]. Phospholipase A2 is a proinflammatory enzyme whose expression is induced by Tumor Necrosis Factor (TNFα) that is inhibited in vitro by the fatty acids produced. In response to this erythrocytic depletion, hematopoiesis releases reticulocytes into the general blood circulation. Moumaris et al. demonstrated that during the first 4 days of the infection of C57BL/6 mice by Plasmodium berghei ANKA, the appearance of a subpopulation of young erythrocytes was invaded preferentially by Plasmodium berghei ANKA, which seems to play a key role in the initiation of the pathology of cerebral malaria. These young erythrocytes observed during the early period of infestation are the result of hemolysis. As a matter of fact, three days after the infestation, the hematocrit begins to decrease. The influx of newly produced blood coming from the spleen and from bone marrow partially compensated the hemolytic activity [28]. Various pathogenic mechanisms of cerebral malaria are proposed: i) the sequestration of infected red blood cells in blood vessels and brain tissue ii) secretion of multiple mediators as cytokines and free radicals iii) alteration of capillary permeability which leads to microhemorrhages and cerebral edema [29-32]. During sequestration, infected red blood cells adhere to healthy red blood cells forming rosettes. This rosetting phenomenon is responsible for severe malaria described in Plasmodium falciparum and Plasmodium chabaudi [33].
Plasmodium falciparum proteins exhibit an affinity for receptors located on the surface of the capillary endothelial cells, that results in sequestration of the parasitized RBC on the endothelium of the deep capillaries. This sequestration appears to be one of the main causes of microcirculatory obstruction and cerebral malaria [34-40]. This interaction involves: i) receptors expressed on the surface of host endothelial cells as CD35, Complement Receptor 1 (CR1), Intercellular Adhésion Molecule 1 (ICAM-1), CD36 and CD31 ii) parasitic adhesion proteins as Plasmodium falciparum erythrocyte membrane protein (PfEMP1) iii) ABO blood group antigens expressed at the surface area of uninfected red blood cells. Blood group O, red blood cells deficient in sialic acid residues or in glycophorin, and RBC variant to spectrin or to band 4.1 in elliptocytosis, are resistant to invasion by Plasmodium falciparum and to severe malaria [41-47]. Sickle cell disease confers host protection to malaria. This protection is a tolerance to Plasmodium through the Nuclear factor erythroid 2-related factor 2/heme oxygenase 1 (Nrf2/HO-1) system. Mice expressing sickle Hemoglobin (Hb) are not susce-ptible to cerebral malaria. Sickle Hb inhibits activation of CD8+ T cells recognizing antigens of Plasmodium [48]. The sequestration of parasitized erythrocytes in the deep capillaries reduced perfusion, inducing a multivisceral suffering. This phenomenon is also observed in the infection of mice by Plasmodium yoelii nigeriensis or by Plasmodium yoelii 17XL [49, 50]. Mice neuro-malarial disease results from an immune response in which cytokines like TNFα increased the expression of adhesion molecules of endothelial cells to monocytes. The result is an accumulation of monocytes in the cerebrovascular system [51-57]. However, the immune response cannot be the sole cause of cerebral malaria; other elements interfere to develop this pathology [28].
4. BIOLOGICAL MEMBRANES
The erythrocytes consist of a plasma membrane enclosing hemoglobin and the enzymes necessary to maintain the integrity of the plasma membrane and gaseous transport of oxygen and carbon dioxide. Circulating red blood cells are anucleated and do not synthesize new surface molecules. The plasma membrane is formed of a continuous double layer of 40% lipid molecules in which 52% various proteins are integrated. An 8% carbohydrates from cell-coat (glycocalyx) covalently bound to proteins and lipids membrane. The carbohydrates are located on the outer side of the cells and play a role in the relationships between cells. The erythrocyte membrane carries the determinants of blood groups. The infected erythrocyte is constituted of a complex system of membranes: i) the trilaminar membrane of the merozoites ii) the membranes of organelles of merozoite as Golgi apparatus, endoplasmic reticulum, primary lysosomes, mitochondria, micronemes, and rhoptries iii) the Parasitophorous Vacuolar Membrane (PVM) and iv) the erythrocyte membrane.

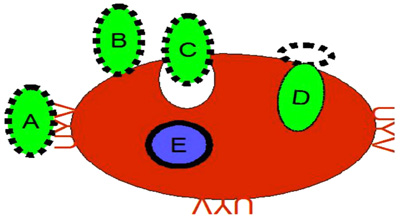
4.1. The Membrane Lipids
A membrane is composed of a bilayer of amphiphilic lipids which consist of phospholipids and glycolipids. The plasma membrane consists of 40% lipids including 55% phospholipids, 25% cholesterol, and 20% glycolipids. Membrane glycolipids include several groups including glyceroglycolipids, glycosphingolipids, and glycophosphatidylinositols. The plasma membrane contains five major phospholipids which are Phosphatidyl Choline (PC), Sphingomyelin (SPH), Phosphatidyl-Serine (PS), Phosphatidyl Ethanolamine (PE) and Phosphatidylinositol (PI). The phospholipids are organized in a double layer. In each layer, the hydrophilic polar heads are directed outwards of the membrane and the hydrophobic aliphatic queues are directed inwards of the membrane. The distribution of lipids in the cell membrane is asymmetric. The majority of phosphatidylcholines and sphingolipids are located in the outer leaflet of the plasma membrane. The majority of phosphatidylethanolamines, phosphatidylinositols and all phosphatidylserines are located in the inner leaflet of the plasma membrane. The outer layer of the erythrocyte membrane contains 80% of the sphingomyelins and phosphatidylcholines compared to only 20% of the phosphatidylethanolamines. The inner layer contained all phosphatidylserines, 80% of phosphatidyl-ethanol-amines, the majority of phosphatidylino-sitols and the remainder of choline phospholipids. Cholesterols are located in equal proportions in the outer leaflet and the inner leaflet of the plasma membrane [58, 59] (Table 1).
Classification | Commentary | References | |
---|---|---|---|
Phospholipids | Phosphatidylcholine (lecitine) |
The phospholipids constitute a solvent for proteins, the nature of their fatty acids then the length and saturation of the carbon chain determine the fluidity of the membrane and condition these physiological properties. Phosphatidylserine and phosphatidylinositol are essential for the invasion of erythrocytes by Plasmodium. During the parasite invasion, proteins secreted by the apical organelle of the merozoite interact specifically with the phospholipids of the erythrocyte membrane. |
[79-86] |
Phosphatidylethanolamine (cephaline) | |||
Phosphatidylserine | |||
Phosphatidylinositol | |||
Sphingomyelin | |||
Glycolipids | Glycosphingolipids (ganglioside) |
Glycolipids play an essential role in the molecular recognition of cell membrane. Upon invasion of the host cells, the proteins of the apical organelle of Plasmodium bind to the erythrocyte via the glycolipids. Plasmodium falciparum proteins located in the microneme of the merozoite bind to erythrocyte membrane via glycosylphosphatidylinositol. |
[87-90] |
Glycoglycerolipids (galactolipide) |
|||
Glycophosphatidylinositols | |||
Cholesterol | Cholesterol contributes to the stability and fluidity of cell membrane by intercalating between the phospholipids. In the plasma membrane, it forms the lipid raft (Detergent-Resistant Membrane (DRM)). DRM is a microdomain rich in cholesterol and sphingolipids, essential for the anchoring of proteins. DRM has an essential role in cellular communication. The mechanisms regulated by DRM are responsible for the uptake of host proteins and maintenance of intracellular PVM in the non-endocytic RBC. | [91-93] |
This asymmetry is linked to a membrane protein, flipase ATP-dependent. This flipase displaces the aminophospholipids (PS, PE) from the outer leaflet to the inner leaflet [60]. Nevertheless, the transfer of choline phospholipids (PC, SPH) occurs according to simple diffusion [61]. The asymmetry of the membrane appears to be important in cell recognition. In fact, if the distribution of the phospholipids is disturbed in the membrane cells, phosphatidylserine can be found in the outer leaflet, then the cells become easily recognizable by the macrophages [62]. Although the physiological function of lipid asymmetry is almost unknown, there are membrane-bound enzymes that utilize this asymmetry, for example, protein kinase C binds to the membrane by interaction with phosphatidylserine being indispensable to its activity. Changes in fatty acid composition by influencing this asymmetry disrupt membrane functions such as transport, receptor-effector coupling, and cell recognition would be a key trigger for cerebral malaria. Glycolipids are mostly located in the outer layer of the membrane, and their glucidic residues are exposed to the surface of cells, suggesting that they play a role in the cell's interactions with its environment [63-65].
Lipids directly affect the physiological properties of membranes and their integrity is essential in the invasion process of the parasite. The intraerythrocytic schizogony of the parasite is accompanied by neosynthesis of a significant amount of Phospholipid (PL) necessary for the biogenesis of these membranes, which leads to a considerable increase in PL content (+500%). After the development of Plasmodium, the PL composition of the parasite appears distinct from the erythrocyte host, with PC and PE being the major Plasmodium PLs [66, 67]. The parasite can synthesize phospholipids, the erythrocyte renews its phospholipids by acylation of plasma lysophopholipids or by exchange with serum lipoproteins, but the parasite as its erythrocyte host can not synthesize fatty acids. A plasma intake is then necessary to the structuring of the new parasitic membranes. Plasmodium and erythrocyte host lack certain enzymes for the synthesis and degradation of the fatty acid [68-70].
Fatty acids are necessary for the development of Plasmodium membranes. The nature of these fatty acids characterized by carbon chain length and unsaturation plays a role in the evolution of parasitemia. The incorporation of fatty acids is very active in the infected erythrocyte. There is undoubtedly a relationship between dietary lipids and the properties of parasitized erythrocytes and their structures; any deficiency of essential fatty acids for the structuring of this complex system of membranes merely limits the multiplication of the parasite [71-73] but it remains to be understood why administration to Swiss mice of large amounts of fatty acids solubilized in tween 20 prevents the multiplication of Plasmodium vinckei petteri or Plasmodium yoelii nigeriensis [74]. The nervous system is the organ that represents the highest concentration of lipids immediately after the fat masses. Dietary fatty acids and more particularly polyunsaturated fatty acids have a direct influence on the composition of the brain membranes and their functioning. During cerebral malaria of mice, polyunsaturated fatty acids deficiency involves neurological abnormalities [75-78].
4.2. The Membrane Proteins
Membrane proteins are determined according to an international nomenclature by reference to their electrophoretic migration. Electrophoresis sizing indicated that the membranes cells contain 7 to 10 major proteins components, which are spectrin, ankyrin, band IV.1a, band IV.1b, actin, aquaporin, band III, and glycophorins. Maturation of reticulocytes to erythrocytes is associated with a decrease in protein synthesis and so are the membrane proteins. The loss of protein synthesizing activity in the maturing reticulocyte involves the loss of polyribosomal material. Reticulocytes also lose hemoglobin and lipids synthesis during maturation [94-99]. There are integral and intrinsic proteins like band III, glycophorins, and aquaporin which are integrated into the phospholipid bilayer of the membrane, detergent or solvent are used to extract them. There are peripheral and extrinsic proteins like spectrin, actin, ankyrin, band IV.1a, and band IV.1b which attach to the lipid bilayer and to membrane proteins. They provide enzymatic, structural, transport, communication, adhesion, and intercellular recognition activities. Membrane skeletal proteins are peripheral structural proteins located on the cytoplasmic surface of the membrane. They are responsible for the shape of red blood cells and represent 60% of the membrane proteins [100, 101].
Reticulocyte Binding-Like family (RBL) proteins play a major role in the mechanisms of invasion of erythrocyte and reticulocyte by Plasmodium. To invade erythrocytes, Plasmodium uses multiple receptors at the level of the spectrin, band 3, actin, glycophorin, band 4.1, band 4.2, aquaporin-1, band 7, and ankyrin. Glycophorin is the major receptor for Plasmodium falciparum. The Duffy glycoprotein is the receptor for Plasmodium vivax [102-107]. Some Plasmodium falciparum binding receptors require erythrocytic sialic acid mostly bound to glycophorins, but others are independent of sialic acid on the erythrocyte membrane [108]. Trypsin cleaves glycoproteins and neuraminidase cleaves sialic acids from sialoglycoproteins. Treatment of red blood cells with trypsin or neuraminidase reduces in vitro invasion of erythrocytes by Plasmodium falciparum [109] (Table 2). The transmembrane glycoproteins of the red blood cells as glycophorin and band 3, are implicated in the Plasmodium falciparum invasion [110]. There is receptor heterogeneity for invasion by Plasmodium, therefore, Plasmodium falciparum can invade RBC deficient in glycophorin A and glycophorin B (MkMk) [111]. Treatment of erythrocytes by pronase inhibits in vitro invasion of erythrocytes by Plasmodium falciparum [112]. The digestion of membrane proteins by chymotrypsin, which cleaves band 3, integrally increases the invasion process suggesting that during the invasion process, digestion of band 3 with protease induces membrane destabilization at the site parasite insertion and leads to the formation of the parasitophorous vacuole [113] (Table 2).
During erythropoiesis, synthesis of the bulk of the spectrin and actin polypeptides is completed before that of the major transmembrane glycoproteins. Synthesis of the glycoproteins ceases before that of several minor proteins found on the inner surface of the red cell membrane, and one of these minor proteins is made predominantly by reticulocytes. The glycoprotein band 3 was weak in the young RBC, which are more susceptible to the invasion by Plasmodium, therefore, it seems that susceptibility to the invasion was correlated to initial interaction parasite-host cells. Upon invasion, the parasites begin to modify the host cells structure making internal and external alterations that enable the parasite to proliferate in the host cells [114, 115]. Several lines of evidence indicate that malaria parasites invade erythrocytes by binding to specific ligand erythrocyte surface [116, 117]. The same erythrocytes membranes proteins are conserved in mammals recognized by different species of Plasmodium. Moreover, the red blood cells of the mice can be invaded by Plasmodium falciparum [118]. Plasmodium lophurae can develop in rodent erythrocytes and Plasmodium berghei can grow in duck embryos [119, 120]. Plasmodium carries receptors on its surface for recognition and invasion of host tissue (RBC, hepatocytes). Plasmodium invasion is achieved by molecular interactions between several parasite proteins and multiple host receptors. Comparative proteomic analysis have identified multiple ligand-receptor and host-cell receptor essential for adhesion and signaling for erythrocyte invasion. The released merozoite recognizes its site and then attaches to the surface of the red blood cell, and reorients itself to put its apical region in contact with the erythrocyte to tie it. A tight junction is formed between the merozoite and the membrane which triggers the invasion process. In this process, an interaction between erythrocyte surface ligands and merozoite surface receptors is essential for the deformability of the erythrocyte plasma membrane and the internalization of merozoite in the red blood cell (Fig. 2 and Table 2). The molecular interactions of Plasmodium with host cells are mediated by thrombospondin structural repeat motif (TSR) expressed at various stages of the parasite's life cycle which are: i) the Circumsporozoite Protein (CSP) and the Thrombospondin-related Adhesive Protein (TRAP) from sporozoites ii) the Merozoite Thrombospondin-Related Anonymous Protein (MTRAP) and the Thrombospondin Related Proteins (TRAMP) from merozoites iii) the CSP TRAP Related Protein (CTRP) from ookinetes [121]. Parasitic proteins such as the Apical Membrane Antigen (AMA), the Rhoptry Neck complex (RON complex), the Erythrocyte Binding-Like family (EBL), the Ring-infected Erythrocyte Surface Antigen (RESA), and the Reticulocyte Binding-Like family (RBL) bind to the erythrocytic proteins such as the glycophorin A, the glycophorin C, the Duffy-Antigen Receptor for Chemokines (DARC), the Complement Receptor 1 (CR1), and the basigin (CD147) allowing the merozoite to enter the erythrocyte by the endocytosis process and forms the parasitophorous vacuole [122-126]. The Rhoptry-Associated Membrane Antigen (RAMA) and the Rhoptry-Associated-Protein (RAP) integrate into the inner leaflet of the plasma membrane then binding specifically PS and PI to initiate the formation of the parasitophorous vacuole [127-131].
During parasitic invasion, erythrocyte membranes interact with multiple parasitic ligands such as the high molecular mass Rhoptry Protein (RHOP-H), the Plasmodium falciparum Rhoptry neck Protein (PfAARP), the Serine Repeat Antigen (SERA), the Mature parasite-infected Erythrocyte Surface Antigen (MESA), the Erythrocyte Binding Antigen (EBA), and the Merozoite Surface Protein (MSP-1). The synthesis of the glycoprotein Plasmodium falciparum Merozoite Surface (PfMSP) proceeds from schizogony to the release of merozoites. During the maturation of the merozoite, PfMSP degrades into several fragments. Anti-PfMSP antibodies inhibit the maturation of Plasmodium falciparum in culture [132-136]. The parasitic factors secreted by the apical organelles of merozoites such as micronemes, rhoptries, and dense granules are used for the invasion of erythrocytes. These ligands include: i) the Plasmodium vivax reticulocyte binding proteins family (PvRBP) and the Plasmodium falciparum reticulocyte-binding protein homologue family (PfRH) present in rhoptries ii) the EBA family and the EBL family present in micronemes [137-139]. During the invasion of reticulocytes by Plasmodium vivax, PvRBPs are essential for the interaction of the parasite with membrane proteins of reticulocytes [140]. Basigin, a multifunctional transmembrane glycoprotein, is the erythrocyte receptor for PfRh5 [141-144].
The spectrin chain forms a hexagonal lattice centered around Actin complex (Ac). The actin complex and the ankyrin complex link spectrin chains to a complex of band 3 and other proteins in the lipid bilayer.
Ankyrin links spectrin to a complex of band 3 (SLC4A1) protein and other proteins in the lipid bilayer. Protein 4.2 binds ankyrin, band 3, CD47 and spectrin. Band 3 protein, an anion-exchange channel, also is associated with glycophorins, blood group proteins Rh, LW and stomatin which interacts with aquaporin, a water channel.
The protein 4.1 links F-actin to spectrin chains and in association with adducin to band 3 (SLC4A1) protein, which is an anion-exchange channel. The actin junctional complex also contains the blood group proteins Kx/Kell and DARC/Duffy as well as glycoproteins and enzymes in the glycolytic metabolon. Stomatin interacts with glucose transporter 1, aquaporin and band 3 protein.
Classification | Comments | References | |
---|---|---|---|
Cytoskeletal proteins | Spectrin | Represents 30% of the total mass of membrane proteins. Formed of two polypeptide chains α and β of molecular masses 280 and 245 kDa respectively, the spectrin filaments interact with the actin and the band IV. Interactions between Plasmodium proteins and spectrin facilitate the entry and exit of merozoites. The exit of merozoites is a process (15-20 hours) using proteases as calpain-1 and perforin which proteolyze the cytoskeletal proteins leading to the dismantling of cellular membrane (Figs. 3, 4 and 5). | [145-157] |
Ankyrine | Cytoskeletal proteins with a molecular mass 215 kDa. Ankyrin interacts with spectrin and band 3. Erythrocytes deficient in spectrin and/or ankyrin, are resistant to Plasmodium invasion (Figs. 3, 4 and 5). | [147-158] | |
Band IV | The band 4.1 (IV.1a, IV.1b) interacts with glycophorin and microfilaments (actin and spectrin). The relative amount of IV.1a increases during aging of the cells. During blood preservation, there is an increase in band II.3 (185 kDa) and band IV.2 (72 kDa). Plasmodium proteins exhibit specific interactions with band IV. | [159-164] | |
Actin | Proteins of the filamentous cytoskeletons (microfilaments) consisting of a double helical chain. Actin and spectrin constitute the filamentous network of the cytoskeleton ensuring the stability of the plasma membrane. Plasmodium proteins specifically bind to spectrin and actin (Figs. 3, 4 and 5). | [148-165] | |
Integral proteins | Aquaporin | Protein pore with a molecular mass of 25 to 35 kD. Water permeable and preventing other molecules (ions) to enter the cell. It is a key molecule in the mechanism of cerebral malaria. An expression threshold of AQP4 is necessary to induce neurovascular pathology. | [166-169] |
Band III | Consists approximately 25% of membrane proteins. It has a role in ion exchange across the membrane. It interacts with band IV.1, band IV.2, glycophorin, ankyrin, and other cytoplasmic proteins. Plasmodium-band 3 interaction plays an essential role in Plasmodium invasion of red blood cells. | [170-174] | |
Glycophorin | Glycophorins (sialoglycoproteins) represent 6% of membrane proteins. Glycophorins carry the majority of sugars and sialic acids. Glycophorin A carries M and N antigens. Glycophorin B carries antigens of blood groups S, s and U. The glycophorins C and D carry antigens of blood group Gerbich. Glycophorins have a major action in Plasmodium invasion of red blood cells. | [174, 175] |



5. PREFERENTIAL RED BLOOD CELLS INVASION BY PLASMODIUM
During hematopoiesis, the erythroblast loses its nucleus and all its cytoplasmic organelles degenerate during maturation to become reticulocyte. The ultimate stages of maturation take place in the peripheral circulation or in the spleen. RBC undergo complex cellular remodeling during the maturation of polychromatic stages to orthochromatic stages. In two to three days, gradually losing ribosomal material and mitochondria, the reticulocyte is transformed into a mature erythrocyte. During the maturation process, reticulocytes lose organelles, water, and membranes. Their polysomes begin to separate into monosomes, then monosomes decrease and disappear [94-98]. Because of the lifespan of the RBC of about 120 days, the reticulocytes constitute a little less than 1% of all RBC in circulation [176, 177].
Susceptibility to parasitic invasion is linked to the terminal stages of the differentiation of RBC. Erythroblast cells are refractory to an invasion. Polychromatic cells are poorly invaded since the ring stage of Plasmodium is less abundant there. Early orthochromatic erythroblasts are the first stages invaded by Plasmodium because the ring stage of Plasmodium is abundant and there are fewer trophozoites there. Late-stage orthochromatic cells and nascent reticulocytes are susceptible to the intracellular growth of the parasite because the intracellular maturation of Plasmodium can be at the trophozoite stages and schizont stages [178]. The parasite uses erythrocyte hemoglobin as a nutritional source for its development inside the erythrocytes [179]. The age classes of RBC constitute one of the constraints of the growth of Plasmodium population. The RBC preference is always enigmatic since the classification of different stages of reticulocyte maturation is limited by technical approaches [180, 181]. Plasmodium species have different preferences for RBC invasion. Plasmodium vivax and Plasmodium ovale have a preference for reticulocytes related to the high density of Duffy antigen [182]. Red blood cells deficient in antigens of blood group Duffy are resistant to invasion by Plasmodium kmowlesi and Plasmodium vivax. Plasmodium vivax and Plasmodium ovale parasitize young red blood cells. Plasmodium malariae would rather parasite old red blood cells. The preference of Plasmodium malariae for mature red blood cells is related to the senescence of markers. For Plasmodium falciparum, its affinity seems to be for RBC of all ages but has an apparent preference for young red blood cells [183, 184]. The aging of red blood cells decreases susceptibility to Plasmodium invasion due to the disappearance of membrane antigens [185, 186]. Most rodent Plasmodium has a preference for young red blood cells.
Electrophoresis of cells membranes and Giemsa-Cresyl staining show that young erythrocytes are more susceptible to invasion by Plasmodium berghei ANKA. This RBC population is similar to that obtained 4 days after mice bleeding. Plasmodium berghei ANKA exhibits a specificity for this young RBC rather than for young reticulocytes or adult RBC. Two days after the infestation of the C57BL/6 mice by Plasmodium berghei ANKA, the young RBC appear. This young RBC increases progressively till it becomes a maximum of 40% of the total red blood cells population the fifth day after infestation [187]. The membrane proteins profile of young cells is different compared to adult erythrocyte. Membrane proteins profiles obtained by coomassie blue coloration show the presence of classical integral and cytoskeletal proteins of erythrocytes (lane 1). This profile is not significantly modified in adult erythrocyte from 4-5 days after infestation of mice or 4 days after bleeding of mice (lane 2, lane 3). The more susceptible the cells to invasion and the young erythrocytes (lane 4, lane 5) show a largely modified protein composition, for example, bands 2-1 (ankyrin) and 4-9 (demantin) are more abundant compared to adult red blood cells. In contrast, the level of glycoprotein band 3 is very weak, so in the recognition invasion process of Plasmodium berghei ANKA for mice, the presence of a glycoprotein band 3 does not seem to be required (Fig. 6). The Giemsa-Cresyl double staining identifies the ribosomes and the parasite Plasmodium berghei ANKA in a different stage of RBC maturation. Observation by light microscopy of blood cells stained by Giemsa-Cresyl shows that the erythrocytes can be divided into four classes as follows: i) The youngest includes cells that stain cytosol polysome ii) The medium age includes cells stained with a medium cytosol monosome iii) The advanced age includes cells stained with a weak cytosol monosome iv) The mature RBC includes cells that stain negatively for Cresyl. The Giemsa-Cresyl demonstrated that young RBC having a preference from Plasmodium berghei ANKA are more susceptible to invasion by Plasmodium berghei ANKA than mature RBC. The Giemsa-Cresyl double staining identifies the parasite among the youngest RBC, the medium age RBC, the advanced age RBC and the mature RBC. The Giemsa-Cresyl locates the Plasmodium berghei ANKA predominates in the RBC with medium age (Fig. 7). The preferential RBC invasion occurs through specific interactions between RBC and Plasmodium [188, 189]. The great majority of total RBC surface carbohydrate include more than 90% of the sialic acid and therefore most of the negative charges of the surface are carried by glycophorins [190]. The merozoite recognition and invasion are sugar dependent, based on the utilization of fluorescent neoglycoproteins at the endoerythrocytic stage of rodent Plasmodium berghei [191]. Differences in membranes cell charge correlated with the oligosaccharide structure linked to glycoprotein could explain the parasite's preference for young rather than old cells.
In-vivo study model “mice - Plasmodium berghei ANKA” shows different membrane proteins profiles of RBC. Reticulocytosis was induced in C57BL/6 mice by removal of 35% of the blood volume by bleeding. The blood containing an average of 25% reticulocytes was harvested 1 day later. Cells were separated over the gradient of Percoll, purchased from Sigma Saint-Quentin Fallavier France, at 600 g for 20 min. In line 1, there is erythrocytes control ; in line 2, there are erythrocytes from 5 days after the infestation of mice ; in line 3, there are erythrocytes from 5 days after bleeding of mice ; in line 4, there are young erythrocytes from 5 days after the infestation of mice ; in line 5, there are young erythrocytes from 5 days after bleeding of mice. The cells were lysed in hypotonic phosphate buffer containing inhibitors of protease as pepstatin and aprotinin. The suspension was centrifuged at 13000 g for 15 min. The membrane was solubilized in 60 mM Tris-buffer pH 6.8, 5% 2-mercaptoethanol, 2% SDS and 10% glycerol, purchased from Sigma Saint-Quentin Fallavier France, then incubated for 3 min at 100 °C. Proteins membranes at 15 µg were separated by 15% SDS-PAGE electrophoresis and stained with Coomassie Brilliant Blue R-250, purchased from Sigma Saint-Quentin Fallavier France [Moumaris et al., 1996].
6. THERANOSTIC PERSPECTIVES ON MALARIA
Different methods of diagnosing malaria have been developed, including parasitological, immunological and molecular biology techniques. Parasitological methods involve microscopic blood smear examination for the detection of Plasmodia. Immunological methods involve examination using immunoassays and flow cytometry techniques to detect Plasmodia antibodies and antigens. PCR methods are more sensitive and specific in identifying Plasmodia species [192, 193]. Vector control by treatment with insecticides as pyrethroids allowed the prevention of malaria, however, the resistance of the anopheles renders this prophylaxis ineffective. Chemotherapy has long been used, with natural antimalarials drugs as quinine extracted from cinchona bark and then with synthetic antimalarials as chloroquine, pyrimethamine, proguanil, mefloquine, and malarone. Chemo-resistance makes these drug treatments less effective; this resistance is mainly through biological membranes and metabolic pathways [194-196]. The malarial vaccine provides protection against infection [197-199]. Several approaches in the development of a malaria vaccine have been considered: i) the parasite dead or attenuated ii) CSP derived antigens to prevent hepatocyte infestation iii) the anti-merozoite vaccine against antigenic proteins of merozoites such as the MSP iv) the anti-gametocyte vaccine to interrupt mosquito transmission and subsequent contamination of other subjects v) the multicomponent vaccine to inhibit the development of the parasite from the sporozoite stage to merozoite stage vi) the vaccines reconstituted by synthesis [200-206]. The host-targeted therapeutics for malaria has been identified in the process used by Plasmodium to invade erythrocytes. Merozoite surface proteins and those secreted by its apical organelles are involved in the process of erythrocyte invasion ; they are vaccine candidates and drug targets against malaria. A malaria vaccine candidate targets PfRh5, MSP-1, AMA-1, and EBA-175 [207]. Invasion assays have been developed for primate malaria. A successful vaccine would be a combination of antigens involved in invasion. Proteomic and transcriptomic data of parasites show that malaria proteins have large polymorphism strategies against the host immune mechanisms [208-210]. The protective polymorphism against malaria is manifested in: i) malaria susceptibility genes as HLA, ICAM, and CD36 ii) structural proteins as hemoglobin and duffy antigen iii) erythrocytic enzymes as G6PD and PK. Erythrocyte mutation of band 3 in ovalocytosis, of ankyrin in spherocytosis, of spectrin in elliptocytosis, or of protein 4.1 have a resistance effect against Plasmodium [211]. Two distinct hosts and several stages of the life cycle make the host-Plasmodium interaction complex, generating molecular variants that exert resistance effects of this host-parasite interaction. The realization of an effective malaria vaccine remains a difficult prospect linked to epidemiology, antigenic variations and genomic polymorphism of the parasite. Vector control, drugs, and transfusion remain the curative and prophylactic treatment in the medium term.
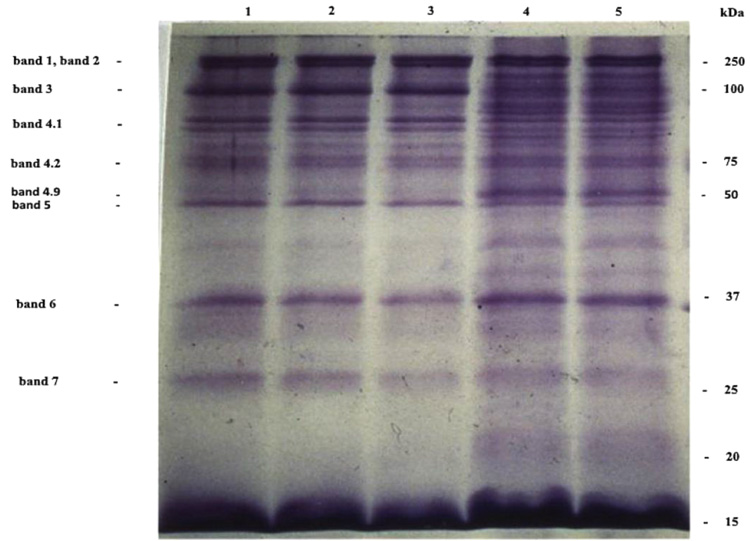

CONCLUSION
Currently, to struggle against malaria there are medicines, the eradication of mosquitoes and the prevention of mosquito bites (mosquito nets). Several drugs are used such as quinine, chloroquine, mefloquine, amodiaquine, pyrimethamine, doxycycline, sulfadoxine, dapsone, proguanil, atovaquone, and artemisinin-based combination therapy. All these drugs are confronted with parasitic drug resistance. Biological membranes have a major role in host cells invasion by Plasmodium. Membrane carbohydrates, proteins, and lipids are essential for the malaria parasite invasion. The preferential cells invasion occurs through specific interactions between cells and Plasmodium. Plasmodium recognizes specific receptor of host cells and enters these cells to develop. Plasmodium invasion is achieved by molecular interactions between several parasitic ligands and multiple host receptors on the biological membranes. At the moment, there is no vaccine against malaria, but there are vaccine candidates in development. The realization of an effective malaria vaccine will be confronted with the complex interactions between biological membranes and malaria parasites.
LIST OF ABBREVIATIONS
Ac | = Actin complex |
AMA | = Apical Membrane Antigen |
AP-HP | = Assistance Publique des Hôpitaux de Paris |
AQP4 | = Aquaporin-4 |
CD | = Cluster of Differentiation |
CD147 | = Basigin |
CR1 | = Complement Receptor 1 |
CSP | = Circumsporozoite Protein |
CTRP | = CSP TRAP Related Protein |
DARC | = Duffy-Antigen Receptor for Chemokines |
DRM | = Detergent-Resistant Membrane |
EBA | = Erythrocyte Binding Antigen |
EBL | = Erythrocyte Binding- Like Family |
GM-CSF | = Granulocytes Macrophages Colony Stimulating Factor |
G6PD | = Glucose-6-Phosphate Deshydrogenase |
GPA | = Glycophorin A |
GPC | = Glycophorin C |
HLA | = Human Leukocyte Antigen |
ICAM | = Intercellular Adhésion Molecule |
IL3 | = Interleukin 3 |
MESA | = Mature Parasite-Infected Erythrocyte Surface Antigen |
MSP | = Merozoite Surface Protein |
MTRAP | = Merozoite Thrombospondin-Related Anonymous Protein |
PbA | = Plasmodium berghei ANKA |
PC | = Phosphatidylcholine |
PE | = Phosphatidylethanolamine |
PfAARP | = Plasmodium falciparum Apical Asparagine Rich Protein |
PfEMP1 | = Plasmodium falciparum Erythrocyte Membrane Protein 1 |
PfMSP | = Plasmoddium falciparum Merozoite surface |
PfRH Family | = Plasmodium falciparum Reticulocyte-Binding Protein Homologue |
PS | = Phosphatidylserine |
PI | = Phosphatidylinositol |
PK | = Pyruvate Kinase |
PL | = Phospholipid |
PV | = Vacuole Parasitophore |
PvRBP | = Plasmodium vivax Reticulocyte Binding Proteins |
PVM | = Parasitophorous Vacuolar Membrane |
RAMA | = Rhoptry-Associated Membrane Antigen |
RAP | = Rhoptry-Associated-Protein |
RBC | = Red Blood Cells |
RBL | = Reticulocyte Binding-Like Family |
RESA | = Ring-infected Erythrocyte Surface Antigen |
RHOP-H | = High Molecular Mass Rhoptry Protein |
RON Complex | = Rhoptry Neck (RON) Complex |
SERA | = Serine Repeat Antigen |
SDS-PAGE | = Sodium Dodecyl Sulphate Polyacrylamide Gel Electophoresis |
TNF![]() |
= Tumor Necrosis Factor |
TSR | = Thrombospondin Structural Repeat Motif |
TRAP | = Thrombospondin-Related Adhesive Protein |
TRAMP | = Thrombospondin Related Proteins |
Nrf2/HO-1 | = Nuclear Factor Erythroid 2-Related Factor 2/Heme Oxygenase 1 |
Hb | = Hemoglobin |
UPMC | = Université Pierre et Marie Curie Paris. |
CONSENT FOR PUBLICATION
Not applicable.
CONFLICT OF INTEREST
The authors declare no conflict of interest, financial or otherwise.
ACKNOWLEDGEMENTS
We thank Monique Abuaf and Suzanne Nazabal AP-HP. We thank Said Youssouf Chanfi UPMC and Bernard Lebeau AP-HP. This work was supported by Association Recherche et Développement Biomédical.